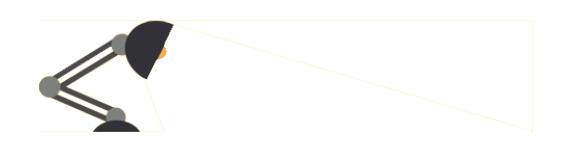
This public number of literature is all interpreted by the author, welcome to contribute!
First author: Shi Zhiqiang, Li Huiping
Corresponding authors: Li Shaochun, Zhu Wenguang
Communication units: Nanjing University, University of Science and Technology of China
DOI: 10.1021/acsnano.0c04620
Full text at a glance
The growth and preparation of two-dimensional materials is the basis of experimental research. Black phosphorus has a folded honeycomb-like structure, which can only be obtained by top-down stripping, but monolayer antimonyene with the same structure has achieved molecular beam epitaxial growth [Z.-Q]. Shi et al., Adv. Mater. 31, 1806130 (2019);Nano. Lett., 20, 8408−8414 (2020)]。 To study the growth mechanism of folded honeycomb antimony, the authors reduced the epitaxial growth temperature to about 350K, thereby freezing the intermediate state during growth. Using scanning tunneling microscopy (STM) observations, the authors found that antimony has two different morphologies, and a uniform fold honeycomb-like structure can be obtained by annealing. Combined with density functional theory (DFT) calculations, the authors determined that the two morphologies before annealing correspond to the folded honeycomb structure (α phase, also known as the black phosphorus phase) and the twisted hexagonal structure (dH, distorted hexagonal structure), and the dH phase after annealing is transformed into a stable α phase structure. Through a combination of experiments and theory, the work reveals a two-step growth pattern of fold honeycomb antimony kinetics, in which a metastable twisted hexagonal (dH) phase is first formed as the coverage of antimony atoms deposited on the substrate increases, followed by a stable α phase under annealing conditions.
Background
Black phosphorus is a two-dimensional material with a folded honeycomb structure, which has great application prospects in optoelectronic devices. So far, the preparation of monolayer black phosphorus is basically through top-down mechanical or chemical peeling method, and it is difficult to grow directly by atomic deposition. The large-scale controllable preparation of materials is generally achieved through a bottom-up method, and the stripping method greatly limits the development of materials in the direction of application.
On the other hand, the monolayer structure of the antimony element (Sb), which is the same main family as phosphorus, and its α phase (black phosphorus phase) has been successfully prepared experimentally by the bottom-up molecular beam epitaxial (MBE) method, and the prepared antimonyene can be stable in air and has unique electronic properties. However, the growth mechanism of α-phase monolayer antimony is still unclear. Studying the growth mechanism of this folded honeycomb-like material not only helps to improve the quality of material preparation, but also has guiding significance for the preparation of materials with similar structures. To this end, the authors used STM to observe the morphology of each stage of growth by controlling the growth conditions, combined with DFT calculations, revealing a kineticly limited two-step growth pattern of folded honeycomb monolayer antimonyene.
Graphic and text analysis
As shown in Figure 1, α phase Sb is a layered material with a folded honeycomb structure, the same as the black phosphorus structure. The lattice of the α phase Sb and the SnSe substrate is highly matched, showing a uniform structure at a growth temperature of >400K, and the presence of molar stripes and the atomic resolution diagram show that Sb maintains a single layer of α phase structure in the substrate.
Figure I. Epitaxial growth of the monolayer α phase Sb on the SnSe substrate. (a) Atomic structure of α phase Sb (top and side view). (b) STM plot of monolayer α phase Sb epitaxial growth on SnSe substrates
However, when the growth temperature drops below ~350K, Sb exhibits very large inhomogeneity on the SnSe substrate (Figure 2a), and STM measurements show that the Sb monolayer has two different heights, namely the raised α phase region and the concave twisted hexagonal structure (dH) region. Continuous annealing at ~350K can lead to a gradual decrease in the area of the dH region of the depression, while the raised α phase area gradually increases, eventually forming a uniform α phase monolayer. The experimental results show that the dH structure of the recessed area is slowly transforming into a α phase structure.
Figure II. Morphological changes of Sb deposited on the SnSe substrate during annealing. (a) Morphology of Sb grown on SnSe substrate at 350 K. (b) Height information measured along the red arrow in figure (a). (c) Topography of annealing at 350 K for 10 min. (d) Topography of annealing at 350 K for 30 min.
To further determine the structure of the recessed region, the atomically resolved STM results (Figure 3) show that the topmost atoms of the recessed region are triangularly arranged and the orientation on the SnSe substrate is chaotic, indicating that this structure does not match the lattice of the substrate. DFT calculations show that it is impossible for a structure that presents such a regular arrangement on an SnSe substrate to have only one layer of Sb atoms. Based on the structural data of the STM, as well as the electronic state information of the STS spectrum, the DFT calculation results show that the dH Sb in the recessed region is a twisted hexagonal diatomic layer structure. The formation energy of this structure on the SnSe substrate is ~59meV/Sb higher than that of the α phase Sb, which is a metastable structure.
Figure III. STM atomic structure diagram of the half-layer Sb. (a-c) Atomic resolution diagram of the native sample. (d,e) Atomic resolution plot after annealing at 350 K for 30 min. Zoom in on part of the upper row to get the bottom row.
The experimental results show that the dH phase Sb will change to the α phase under annealing conditions. To explain this process, the DFT calculation uses the method of climbing elastic bands to calculate the barrier of the phase transition. In the traditional path, a pair of adjacent atoms in the two layers of the Sb lattice of the dH structure flips up and down without bond breakage, and the barrier is 525meV/atom. But the authors found that a path with a lower barrier can be transformed from the dH phase to the α phase, first a pair of adjacent atoms in the dH structure move laterally, misalign each other, undergo the fracture of the bond and the formation of a new bond, forming an intermediate state, and then the lattice of the intermediate state changes towards the α phase lattice, at this time the total barrier is 241meV/atom. In addition, calculations show that by forming a local α phase nucleus in the dH phase and then slowly growing from the α phase nucleus, the barrier can be reduced to 145meV/atom. The calculations show that the transition from the dH phase to the α phase is easy, while the reverse process is more difficult.
Figure IV. Dynamic path map of the transition from dH structures to α structures. The gray line represents the path of the traditional direct transformation, and the red line is the path through an intermediate transformation.
Summary and outlook
By controlling the epitaxial growth temperature of the α phase Sb, the authors observed that a half-layer of dH phase appeared before the formation of the α phase, and the combination of experiments and calculations revealed the two-step growth pattern of this folded honeycomb-like two-dimensional material. In view of the fact that another phase of phosphorene, namely blue phosphorus, has been experimentally prepared from the bottom up, by selecting a suitable substrate, controlling the speed and temperature of atomic deposition, the growth mode proposed by the authors has guiding significance for the bottom-up preparation of black phosphorus. In addition, through more precise control of experimental conditions, more refined analytical methods, the kinetic processes behind them can be further determined, providing a basis for future large-scale material preparation.
Corresponding Author Introduction
Professor Li Shaochun, a professor at the School of Physics of Nanjing University, has long been engaged in experimental research on low-dimensional systems, exploring its possible application value in the field of devices from three aspects: preparation, characterization and regulation. Mainly using high-resolution scanning tunneling microscopy technology and molecular beam epitaxial method, it is mainly used to grow in situ high-quality monolayer films and other low-dimensional materials, characterize morphology, electron/spin ground state and excited state in real space and energy space at the single atom scale, and find and discover novel quantum phenomena. His research interests focus on topological material systems, molecular functional materials, energy materials, metal/semiconductor table interfaces, oxides, and other low-dimensional composites and novel quantum systems.
Email:[email protected]
Professor Zhu Wenguang, a professor at the University of Science and Technology of China, is mainly engaged in theoretical research on the growth mechanism and physical and chemical properties of low-dimensional nanostructures and new functional materials. By using first principles to calculate electronic structure calculations, molecular dynamics simulations, Monte Carlo simulations and other multi-scale simulation calculation methods, the structural stability and growth mechanism of materials are studied from the atomic scale, and how to obtain structures or materials with certain characteristics by controlling the kinetic process of material growth, and then use first-principles electronic structure calculations to study the physical and chemical properties of materials such as electronic transportation, magnetism, optics, topobicity of electronic structures, surface catalysis, etc. It also strives to design new functional materials or structures with practical application value from theory, focusing mainly on clean energy, spintronics and other fields related to future microelectronics.
Email: [email protected]
Literature source
Zhi-Qiang Shi, Huiping Li, Qian-Qian Yuan, Cheng-Long Xue, Yong-Jie Xu, Yang-Yang Lv, Zhen-Yu Jia, Yanbin Chen, Wenguang Zhu, and Shao-Chun Li. Kinetics-Limited Two-Step Growth of van der Waals Puckered Honeycomb Sb Monolayer. ACS Nano 2020.
https://pubs.acs.org/doi/10.1021/acsnano.0c04620.