The following article is from Nature Portfolio, author of Nature Portfolio.
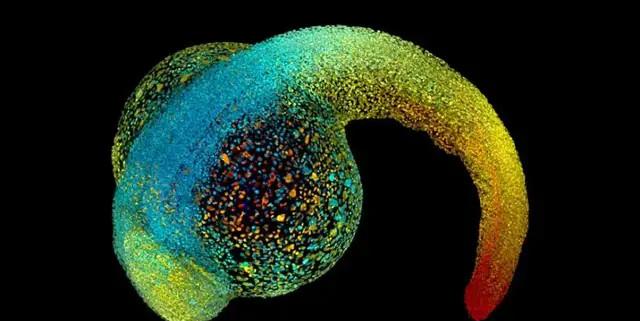
The developing embryo — the zebrafish in the picture — grows up relying on physical forces to shape its own shape | Source: Philipp Keller/HHMI Janelia Research Campus
Introduction
Scientists are studying the role of mechanical forces in the human body — from embryo to adulthood.
Written | Amber Dance
At the beginning of life, an embryo, regardless of front and back, head and tail, is a simple mass of cells. But soon, the smooth pellets of cells begin to change, the fluid gathers to the center of the plexus, and the cells flow like honey, occupying their place in the future body. The cell layer folds like origami to build the heart, intestines, and brain.
None of this would have happened without squeezing, bending and pulling forces to shape the developing animal. Even after adulthood, its cells continue to respond to thrusts and pulls from each other and from the environment.
Amy Shyer, a developmental biologist who studies organ formation at Rockefeller University in New York, says patterns of body and tissue formation remain "one of the most important, but little-known, problems of our time." For decades, biologists have focused on the way genes and other biomolecules shape the body, largely because the tools for analyzing these signals are readily available and have been advancing. Mechanical forces, by contrast, receive much less attention.
Xavier Trepat, a mechanobiologist at the Catalonia Institute for Bioengineering in Barcelona, Spain, says that studying only genes and biomolecules is "like writing a book with only half the alphabet."
Over the past 20 years, more and more scientists have begun to pay attention to the importance of mechanics in various stages of development in organs and organisms. Researchers began to define the mechanisms by which cells sense, respond, and generate force. They do this by inventing custom tools and developing tricks that combine lasers and micropipettes, magnetic particles, and custom microscopes. Most researchers are using cells or tissues grown in petri dishes to probe mechanical signals, and only a few groups are studying entire animals, which tend to find mechanisms that behave differently from isolated tissues. Roberto Mayor, a developmental biologist at University College London, says these in vivo studies have many challenges — such as measuring tiny forces in complex tissues — but they are critical to understanding the role of force in sculpting life.
A small number of determined scientists have risen to the challenge and have observed the key forces of shaping organisms – from the initial stages of embryonic existence to the late life of disease. In the future, this information may lead to better interventions for problems such as infertility or cancer.
"As long as there is a shape, it is powerful." Thomas Lecuit, a developmental biologist at the French Institute of Developmental Biology, says.
Powerful from the start
Before the embryo can take shape, it must break the symmetry of the smooth cell mass. After learning about the manipulation of this process by genes and chemistry, scientists now have a better understanding of mechanics. Jean-Léon Ma tre, a biologist at the Curie Institute in Paris, says: "Little by little, the role of mechanical forces in the developmental process is gradually revealed. For example, physical properties such as fluid pressure and cell density are key before, after, head, and tail of mammalian embryos.
Ma tre's team studied how the initial clumps of cells that make up the earliest mouse embryos formed a huge, fluid-filled cavity. As this cavity fills, the cells that are about to form the fetus are pushed aside. This was the first event to break the symmetry, ensuring that the embryo implanted correctly into the uterine wall and determining which side of the embryo was the back and which side was the abdomen. But it is unclear how the embryo generates and localizes the cavity (see "Developmental Pressure").
Figure 1 Source: Reference 2
When Ma tre's team imaged the process in detail, they found something unexpected. "We saw these little blisters, and they formed between cells," Ma tre said, "and they were fleeting — if the imaging wasn't fast enough, they would be missed." "The fluid in these blisters comes from the fluid around the embryo [1], which is forced to enter the interior due to the higher concentration of water molecules on the outside. The team then saw water flowing from a single blister to a large cavity, which Ma tre thought might be through a gap between cells.
The researchers confirmed how this happened by looking at proteins that cross the cell gap, which come into contact with each other to hold the cells tightly together [2]. When blisters appear, these adhesion proteins appear to rupture as the cells are pushed away. Cells with fewer adhesion proteins are more likely to be separated.
Ma tre said this is the first time it has been observed that a pressurized fluid can shape an embryo by disrupting the connections between cells. Why do embryos shape themselves by forcing cells to separate? "This approach seems inefficient and risky," he said. The best reason he could think of was that the strategy evolved not because it was the best, but because it was good enough. The team is currently working on human cells, and he hopes that further understanding of embryo mechanics will help some IVF clinics determine which embryos will achieve successful conception.
In subsequent development, the embryo breaks the symmetry in one direction, differentiating into head and tail. Otger Campàs, a biophysicist at the University of California, Santa Barbara, tracked the growth of the tail in Danio rerio embryos [3]. His team measured the forces involved by injecting oil droplets loaded with magnetic nanoparticles into the interval between cells. The team then applied a magnetic field to deform the droplets in order to measure how the tissue responded to the thrust.
Figure 2 To push and pull the cells of this zebrafish embryo, the researchers used a magnetic field to deform a magnetic droplet (yellow) | Image credit: Alessandro Mongera and Ogger Campàs, University of California, Santa Barbara
They found that the tip of the tail is in what physicists call a "fluid" when it develops—cells flow freely and the tissues are highly deformed when pressed. The farther away from the tail end the tissue is also harder. Campàs recalls, "We knew it was hardening, but we didn't understand the mechanics. ”
There was nothing between cells that increased hardness—there were no molecules that could make up the structural matrix—but when the researchers measured the spacing between cells, they found that the distance was larger in the wet and soft tail tip, and the closer to the head, the smaller the distance [4]. When cells come together, the tissue also hardens. Campàs likens this transition to the process of packing coffee pellets: coffee pellets can be poured freely into bags, but packed too tightly, and the full bags are as hard as bricks. He intends to investigate whether this mechanism is the basis for the formation of other embryonic structures, such as limb buds.
Make the heart and brain
Once the developing embryos are arranged, the various organs begin to form. Timothy Saunders, a developmental biologist at the National University of Singapore, said: "Fundamentally, we don't know much about the mechanisms by which any one internal organ is formed. (He noted that the gut is an exception.) )
That has changed. For example, Saunders' group studied heart formation with embryos from Drosophila, a fruit fly. A key event is that two pieces of tissue come together to form a tube, which eventually becomes the heart. Each piece of tissue contains two types of cardiomyocytes. These tissues must be combined correctly, like zippers, mirror paired, to get a healthy heart. "We often see combinations mismatched and then corrected," Saunders said, "what caused this correction?" ”
It turned out to be a force that came from the heart cells themselves. The protein, called myosin II, is a close relative of a protein that contracts muscle cells, and is known to flow from the center of each cell to the edge of the cell during the zipper. Shaobo Zhang, who was a graduate student at the time — now preparing for a postdoctoral position at the University of California, San Francisco — wondered if myosin would produce the reaction force of these paired cells to bind, breaking the connections between mismatched types.
To test his theory, Zhang used a laser to cut open pairs of cells. The cells detached quickly, like a tight rubber band suddenly being cut off. Saunders said: "We can see a nice recoil. But when the team cut open the cells that lacked myosin II, "nothing happened." Myosin is like pulling a rubber band with a finger, creating a reaction force of the connection from the inside [5], disconnecting the connection between the mismatched cells and giving them a chance to find the right pair.
Researchers at the University of Cambridge in the United Kingdom found in embryos of Xenopus Xenopus that simple cell proliferation can also send signals to instruct cells to properly self-arrange. A team led by physical biologist Kristian Franze has found that as eye-brain connections gradually form, neurons in the eye cause their axons (axons, which are long synapses that neurons use to contact each other) along paths defined by the different hardnesses of brain tissue. In the developing brain, the axons of the eye follow the softer tissues to the center [6].
To determine when and how that path is formed, the team crafted a microscope. Using this microscope, it is possible to measure the hardness of the tissue using tiny probes while observing processes in vivo [7]. Franze, who heads the Institute of Medical Physics and Microstructure Engineering at the University of Erlangen-Nuremberg, said they saw the hardness gradient appear about 15 minutes before the axon arrived and extended along it.
How do hardness gradients come about? Like the developing zebrafish tail, the harder tissue in the frog's brain appears to contain higher density cells. When the team prevented cells from dividing in the developing embryo, the hardness gradient no longer appeared and the axons could not find their path. Stuffing space with cells appears to be a quick and effective way to direct the nervous system into connections.
Constant stress
Mature animals must also compete with force as they continue to grow or cope with disease. For example, when the body swells, the skin also grows to cover it. Surgeons take advantage of this in breast reconstruction, which requires more skin to cover the implant. First, they insert a "balloon" and inject salt water over a few months to gradually enlarge it, stretching the original skin until enough new skin has grown before undergoing a second surgery.
But how do skin cells respond to this stress and proliferate? Stem cell biologist Mariaceleste Aragona solved this problem in collaboration with Cédric Blanpain while a postdoctoral fellow at the Vrije Universiteit Brussel in Belgium. She implanted a self-expanding hydrogel globules under the skin of mice [8]. As the hydrogel absorbs the liquid to a final volume of 4 ml, the skin also stretches around it. Within a day of implanting the hydrogel, Aragona saw stem cells under the outer layer of the skin begin to proliferate, providing the raw materials to differentiate into new skin.
But not all stem cells can respond to this stretch and proliferate — only one previously unknown subset will begin to export new stem cells. "We still don't know why," says Aragona, who currently works at the University of Copenhagen. Blanpain added that elucidating that this system promises to crack methods that promote skin growth for surgical reconstruction or wound healing.
The mechanical properties of tissues also play a role in abnormal cell growth, such as cancer. Trepat said: "Solid tumors are harder than normal tissue. Part of the reason, he said, is due to the excess presence of a fibrous mesh called the extracellular matrix around the cells, and also because the cancer cells themselves are proliferating.
Trepat explains that "hardness increases the malignancy of cancer cells," and if scientists can understand why, they may be able to design therapies that alter these physical properties and reduce the risk of cancer.
In a related study, researchers at Rockefeller University have confirmed that mechanical forces can explain why some skin cancers are benign and some are malignant. Skin stem cells produce two types of cancers: basal cell carcinomas that do not spread outside the skin and invasive squamous cell carcinomas. Both of these squeeze the underlying basement membrane, a layer of structural proteins that separate the outer layer of the skin from deeper tissue. Benign basal cell tumors rarely cross the basement membrane, but aggressive squamous cell tumors often escape, wander through the vasculature, and colonize other parts of the body (see "Mechanisms of skin cancer").
Figure 3 Source: Reference 9
Stem cell biologists Elaine Fuchs and Vincent Fiore found in their study of mouse skin that benign cancers form a thicker, softer basement membrane that contains tumor cells as if they were wearing gloves when squeezed downwards. Aggressive tumors, on the other hand, form a thinner basement membrane.
The force from above also helps to escape the invasive tumor. Squamous cell carcinoma forms a hard layer of differentiated skin cells called keratin beads. By squeezing the top of the tumor, keratin beads help the tumor break through the fragile basement membrane, like a punch breaking glass [9].
Fuchs said that before conducting the study, the researchers had thought that these differentiated skin cells with fixed characteristics did not produce mechanical force. She said: "I think that's the biggest surprise. ”
Next, Fuchs and Fiore plan to study how cells perceive these mechanical forces and how they convert them into gene expression programs that could generate more basement membranes or promote differentiation.
Alan Rodrigues, a developmental biologist at Rockefeller University, says the relationship between forces and genes is a key issue. It's not just a skin cancer problem. He said: "The deep problem in mechanics is actually the question of what force has to do with molecules. ”
Others are also studying the relationship. Lecuit said: "It's not a one-size-fits-all approach: 'Everything is a function of genes' or 'Everything is a force', but an interesting conversation between the two," Lecuit said. ”
The original article was published under the headline The secret forces that squeeze and pull life into shape in a news feature section of Nature on January 13, 2021.
Resources:
1. Schliffka, M. F. et al. Preprint at bioRxiv https://doi.org/10.1101/2020.09.10.291997 (2020).
2. Dumortier, J. G. et al. Science 365, 465–468 (2019).
3. Serwane, F. et al. Nature Methods 14, 181–186 (2017).
4. Mongera, A. et al. Nature 561, 401–405 (2018).
5. Zhang, S., Teng, X., Toyama, Y. & Saunders, T. E. Curr. Biol. 30, 3364–3377 (2020).
6. Koser D. E. et al. Nature Neurosci. 19, 1592–1598 (2016).
7. Thompson, A. J. et al. eLife 8, e39356 (2019).
8. Aragona, M. et al. Nature 584, 268–273 (2020).
9. Fiore, V. F. et al. Nature 585, 433–439 (2020).