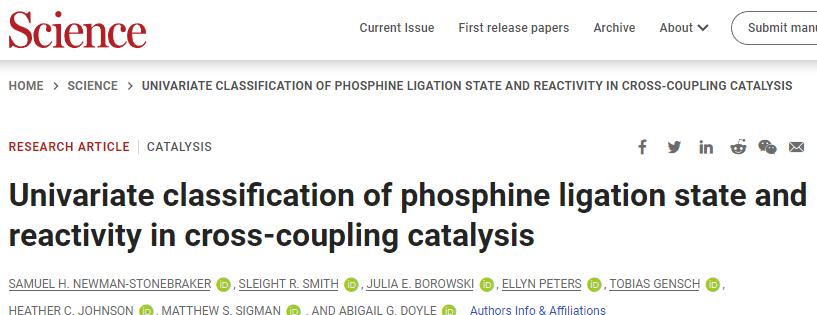
First author: Samuel H. Newman-Stonebrakert, Sleight Smith
Corresponding author: Abigail G. Doyle, Matthew Sigman
Communications: Princeton University, USA, University of Utah, USA
DOI: 10.1126/science.abj4213
<h1>Background</h1>
The complex relationship between chemical structure and reactivity can be understood by using data-driven modeling. For many reactions, there is a constant dependency between descriptors (mathematical methods of describing subunits or molecular wholes) and chemical reactivity, however, some processes exhibit reactive cliffs, where the criteria or thresholds of a given descriptor must be met in order for a reaction to occur. Transition metal catalytic cross-coupling is a relevant case study for identifying reactive cliffs, which are very important in synthesis due to their wide range of applications in the synthesis of drugs and materials. In addition, the success of these reactions is highly dependent on auxiliary ligands, of which monodondine is the most commonly used ligand. A number of tools have been developed to quantitatively describe the different spatial and electronic properties of monodondylar phosphine for correlating structural-reactive trends in cross-coupled datasets. However, in some cases, seemingly similar ligands produce essentially different responses, suggesting that there is discontinuity in the ligand response.
<h1>Highlights of this article</h1>
● According to whether the metal is preferentially located in one or two phosphine (i.e., catalyst connection state), the authors divide the cross-coupling datasets of nickel and palladium catalyzed into active and inactive regions.
Although %Vbur describes the spatial body of any given ligand structure within the metal center 3.5Å, %Vbur(min), a previously unexplored descriptor variant, quantifies the energy of all ligands to reach the smallest %Vbur of conformations, and can divide datasets of similar thresholds into active and inactive regions.
● This analysis reveals non-intuitive trends in organometallic chemistry, which can be used as an important mechanism tool for understanding and predicting the structure, reactive relationship, and catalyst connection state of monodonium in cross-coupling catalysis.
<h1>Graphic and text analysis</h1>
The success of transition metal-catalyzed cross-coupling reactions depends largely on the properties of the auxiliary ligands, the most common of which are mono-phosphine (Figure 1A). Given the existence of thousands of unique structural examples, chemists have developed a number of tools to quantitatively describe the different spatial and electronic properties of monodonium, including Tolman cone angles, solid angles, solid angles, and buried volume percentages (%Vbur) (Figure 1B). Using the organophosphorus (III) descriptor library recently developed by Gensch et al. (kraken), which has nearly 200 conformational representative descriptors for thousands of mono-phosphine (Figure 1C), the authors were able to divide 11 nickel and palladium-catalyzed cross-coupling datasets into active and inactive regions based on metal preference located in one or two phosphine (i.e., catalyst connection states). This analysis can be used as an important mechanism tool for understanding and predicting the structure-reactivity relationship and catalyst ligation state of monodonium in cross-coupling catalysis (Figure 1D).
Figure 1 Study the reactivity of phosphine ligands with structural descriptors.
Based on the 19 phosphines originally studied in Doyle Labs, the authors added 15 additional ligands to cover the entire %Vbur range (Reaction I; Figure 2A). Using this data, the authors attempted to determine the relationship between the kraken spatial descriptor and the reaction performance. For each reaction, the yield was assessed against a representative descriptor in the library; e.g., cone angle, Boltzmann mean %Vbur [%Vbur (Boltz)] of the ligand conformational system, and the library conformation [%Vbur (min)] with the smallest buried volume (Figure 2B).
Figure 2 Study on phosphine spatial parameters in nickel catalytic dataset.
Next, the authors studied the subset of ligands (28 phosphines) used in the nickel-catalyzed SMC reaction to determine the connection state by spectroscopy. LnNi (4-fluorobenzaldehyde) was chosen as the platform because its three nmR (1H, 19F, 31P) provides in situ connection state at the center of the metal. Reading of Ni(COD)2 (COD, 1,5-cyclooctadiene) reaction with aldehyde and biequirephosphine (reaction VI; Figure 3) (Figure 3A). Depending on the observed spectra, each ligand is designated to form an L2Ni or L1Ni complex. The results of these experiments are then evaluated using spatial features in the library to determine which experiments divide the ligands into regions with similar junction states (Figure 3B).
Figure 3 Ligand state study.
Next, the authors attempt to understand the structural importance of %Vbur(min) through crystallography and computational studies (complexes 1 to 4; Figure 4A). For L1Ni-forming P(t-Bu)3, the %Vbur value of the lowest energy conformation and the Boltzmann weighted average of all conformations (38.7% and 39.5%, respectively) were significantly higher than the values of other L2Ni-forming ligands, and even higher than the %Vbur (Boltz) (36.3%) of L1Ni formation P(t-Bu)3. However, the X-ray crystal structure of [P(i-Bu)3]2Ni(2-naphthaldehyde)(5; Figure 4B) confirms that the two phosphines bind to Ni, and the measured %Vbur values are 29.0% and 32.2%, respectively, almost matching the %Vbur(min). Using Cy2P(t-Bu) as a reference, the ligand dissociation free energy (ΔGdissoc) of 20 L2Ni (benzaldehyde) complexes was calculated with DFT (Figure 4C).
Figure 4 Mechanism study of ligand state threshold %Vbur(min).
Next, the authors generated datasets of two palladium-catalyzed SMCs (reactions VIII and IX; Figure 5A) with aryl halides, including a reaction coupled to a steric hindrance, and a dataset of Buchwald-Hartwig amination (reaction X; Figure 5A). For both SMCs, the classification tool shows that the threshold is approximately %Vbur(min) and the active region appears above this value, indicating that effective catalysis requires larger ligands defined by %Vbur(min) (reactions VIII and IX; Figure 5B).
Figure 5 Threshold analysis of palladium-catalyzed cross-coupling reactions.
Figure 6 summarizes the thresholds and directivity of each dataset studied in this paper. The comparison of Ni and Pd catalytic reactions reveals several interesting mechanistic features of Ni and Pd catalytic reactions, as well as the more general phosphine spatial effect. First, ni and Pd-catalyzed aryl halide SMC reactions (reactions II to V, VIII, and IX) have opposite threshold directions, indicating that the two metals have different connection state requirements for the reaction. As a result, the classification workflow provides a way to compare nickel and palladium and elucidate the orthogonal ligand design principles typically required for each metal.
Figure 6 Overview of reactivity thresholds.
Original link:
https://www.science.org/doi/10.1126/science.abj4213