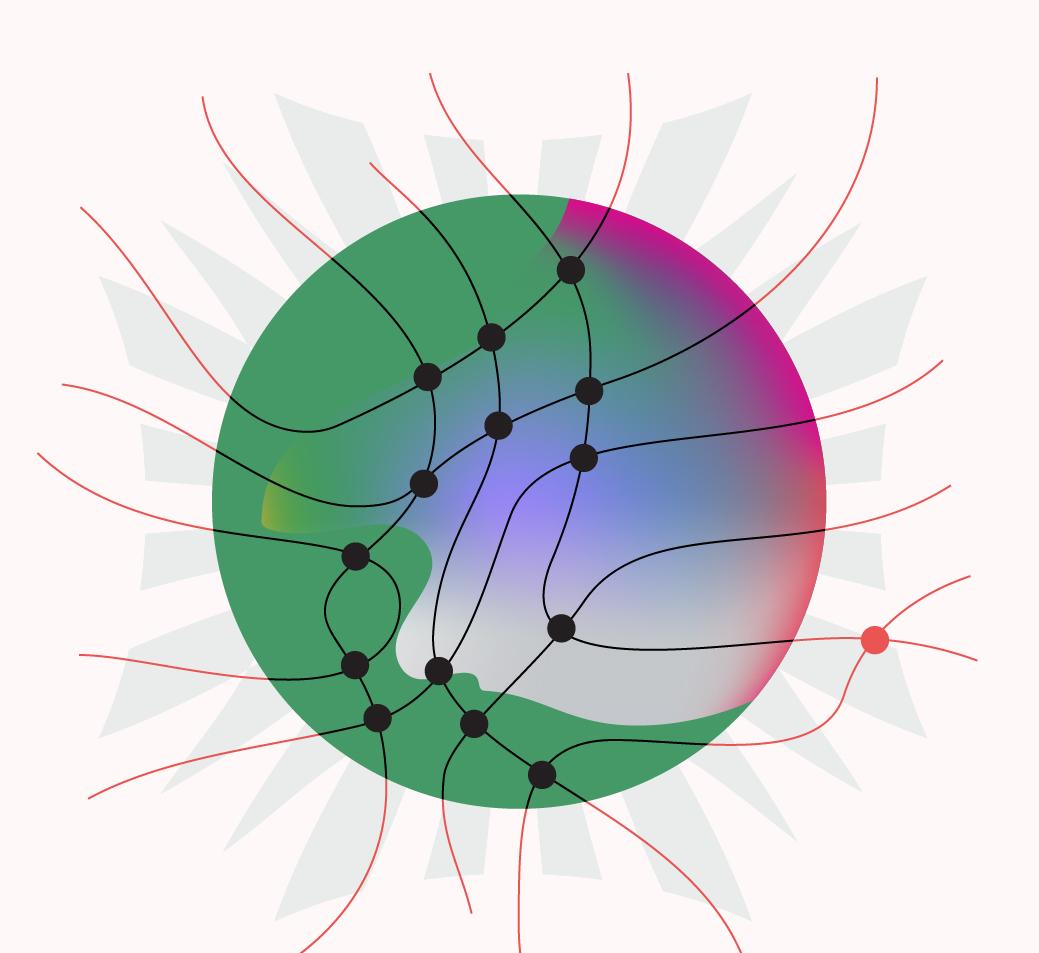
<h1 class="pgc-h-arrow-right" data-track="1" >What is the Standard Model? </h1>
The universe is made up of tiny fragments of matter. Physicists call these fragments particles. As far as we know, there are many different types of particles that cannot be further decomposed. They are the most basic objects in the universe. Physicists call these elementary particles. You may already know some of them, like that an electron is an elementary particle.
In addition to this, there is a force between these elementary particles, and they can attract or repel each other. Under the right conditions, the two particles may even annihilate each other, leaving only energy behind. These forces are called "interactions." Similarly, you probably learned a basic interaction in high school, electromagnetic forces.
So what is the Standard Model? You may have seen this term in popular science books. If not, I will explain everything from the beginning in this article. Physics is about creating "models". A model is usually a mathematical equation that is constructed from a set of observations. Based on a model, we use calculations to predict the occurrence of something or the presence of a particle.
The Standard Model is one such model. It is a theory that currently describes all known elementary particles and their interactions. It is encoded in a Lagrange function. It's not hard to write out the Standard Model explicitly. Its mathematical framework is based on the theory of relativity quantum field (QFT). As I wrote in previous articles, quantum field theory is a mathematical framework for turning abstract fields into particles and other observable quantities.
The Standard Model in its current form
Overall, the Standard Model is one of our most successful physical theories to date, and there is little controversy about that. I'm going to talk about the history of the Standard Model in this article because I think it makes it easier for us to understand the model in its current form. Due to the amount of content, this article will only cover the first half (by 1950)
<h1 class="pgc-h-arrow-right" data-track="8" > radiation and electrons </h1>
The Standard Model covers two different types of important concepts in physics. The first is the particle, the basic component of matter. The second, and equally important, is interaction. Interactions describe the forces between subatomic particles discussed in the Standard Model.
Before the 20th century, there were only two known interactions, gravity (gravity) and electromagnetism. Newton and Maxwell, both mathematicians from Cambridge University, who have studied these interactions, have made important studies of these interactions. The motion of the Moon inspired Newton's study of gravity, and Newton outlined the use of calculus in Principles. Maxwell proposed the theory of electromagnetic waves in The Dynamic Theory of Electromagnetic Fields. He proposed that light itself is an electromagnetic wave. He also calculated the speed of light, the speed of electromagnetic waves. The speed of light is something that Einstein later used to formulate special relativity.
At this point, the "discreteness" of matter had not yet been discovered, and it was not even clear that matter was made of atoms. What drives physics to continue is the discovery of different types of radiation. In 1896, Marie Curie and Pierre Curie, as well as Becquerel and Rutherford, conducted research on radioactivity. This form of radioactivity is made up of different particles emitted from the nuclei of different atoms.
They found three types of radiation, each represented by a different Greek letter α, β, and γ rays. α rays are the nuclei of helium, β rays are just electrons, and γ are light. α and β decay is a hint that gravity and electromagnetism are not the only two types of interactions. We now know that these decays come from strong and weak interactions, respectively. Together with the electromagnetic force and gravity, the strong and weak interactions together describe the four fundamental forces of nature that we have already observed. The weak interaction is responsible for the radioactive emission of the nucleon. Strong forces are responsible for combining quarks to form neutrons and protons.
J· J. Thompson used cathode ray devices to measure electron m/e.
Later, in 1897, J.J. Thompson was one of the first to use cathode ray experiments to measure the properties of electrons. He calculated the ratio of the electron's charge to mass. Years later, it was his son who used diffraction experiments to observe that the electron's behavior was a kind of wave. This diffraction experiment is the first step in understanding that particles can move like waves at the same time.
< h1 class="pgc-h-arrow-right" data-track="15" > further development of protons and electrons </h1>
Even after the discovery of electrons, there are still more mysteries to be solved about the theory of light. For example, the photoelectric effect and the ultraviolet catastrophe problem show that light behaves as a discrete energy packet rather than pure electromagnetic waves. These discrete energy packets are called quanta, and we now call them photons.
From 1900 to 1930, these observations provided inspiration for the development of quantum mechanics. In the process, Einstein theorized that the speed of light is like the "limit of the universe's speed", which is constant no matter what reference frame it is in. This observation led to what is now known as special relativity. In a seminal paper entitled "On the Electrodynamics of Moving Objects" published in September 1905. Today, this theory is my favorite. It has been observed in countless experiments, proving some strange phenomena. Length contraction, time dilation, relativistic mass, universal speed limits and mass-energy equivalence, among others.
In the early 20th century, Rutherford, Marsden, and Geiger conducted a series of scattering experiments that resulted in models of atoms. A scattering experiment is any experiment that shoots one particle into another particle or material. These experiments confirm that, indeed, that most of the positive charge of an atom is tightly concentrated in its center. We now know that atomic nuclei are made up of subatomic particles called protons and neutrons. At this time, however, there are still many questions about what protons are.
Later, in the case of particles found in the nucleus of atoms, there were further developments in both mathematics and theory. In 1919, Aston discovered the bias of the "law of integers" and explained it with isotopes. Isotopes are elements in the nucleus of an atom with the same number of protons and different numbers of neutrons. The law of integers is a theory proposed in the 19th century that the weight of an element is an integer multiple of a hydrogen atom. This means that protons exist as elementary particles.
During that decade, cosmic rays were discovered through cloud chambers, which was also an important experimental development. The cloud chamber is a steam chamber from which the trajectory of the particles can be seen.
Cloud chamber images are used to prove the presence of positrons,
In the 1920s, Bossey, Albert Einstein, Dirac, and Fermi came up with the concept of quantum statistics. Quantum statistics is a set of rules that define the behavior of physically indistinguishable particles when they are in the same position. It speculates that particles are divided into two types: bosons and fermions, depending on their spins. Bosons have an integer spin, and bosons can be together and occupy the same energy state. Fermions have semi-integer spins like 1/2 and follow the Pauli incompatibility principle, which states that two or more fermions cannot be in the same quantum state at the same time. The 1940 spin statistics theorem proposed that all particles are either bosons or fermions.
<h1 class="pgc-h-arrow-right" data-track="23" > quantum field theory and positron </h1>
During this time, we also saw the development of quantum field theory, mainly Heisenberg and Dirac. Quantum field theory is a mathematical tool that holds that the fundamental component of nature is the field. A field is simply a function that assigns a number to a specific point in space and time. So, for example, temperature is a field, and at each point in space, a number can be given. The unit of measurement for this number is Fahrenheit or Celsius. After our first construction of the field, we quantized them into particles. Quantization is the mathematical process of interpreting the vibrations of a field as particles themselves, and then predicting what will happen when the particles interact.
Paul Dirac.
The first attempt to describe the dynamics of electrons in terms of quantum theory was Dirac. Dirac described electrons, but also predicted the existence of positrons (interestingly, he initially thought it was protons). In 1931, Dirac made this prediction about positrons. He also mentioned this in his introduction to his paper on monopoles. Soon, in 1932, Anderson discovered positrons in experiments. Anderson was at Caltech at the time, and in London, Blackett also discovered positrons. This location was observed by finding particles reflected in different directions in the magnetic field in the cloud chamber.
In addition to electrons, Pauli predicted neutrinos in 1930. Chadwick discovered neutrons in 1932. In 1934, Fermi proposed the theory of weak interactions to explain β decay.
< h1 class="pgc-h-arrow-right" data-track="28" > strong interactions and developments in quantum electrodynamics (1930s to 1950s</h1>).
In 1934, Hideki Yukawa proposed the theory of strong interactions. The theorization of this force is intended to explain the force that makes nucleons such as protons and neutrons consistent in atoms. In general, since protons are positively charged, we expect them to repel each other. However, we know from Rutherford's experiments that this is not the case and that there must be other, stronger forces to bind the nucleons together. The strength of this force was originally quantified using the Yukawa potential, a hotly debated equation that physicists believe would "outweigh" the repulsive force of an electron's magnetic pulse.
Yukawas was originally used to quantify the strength of the force that holds protons together.
Yukawa proposed a type of particle called a π meson, which are scalar media with strong interactions. He made calculations and calculated the intensity of the interactions. As the distance increases, this force drops sharply to zero because the range of strong interactions is very short. He estimated the mass of the proton to be 100 MeV. With this mass, strong interactions become very important over very short distances.
After this prediction, people began looking for protons, and in 1936, Anderson discovered what we now call μ mesons. the mass of μ mesons is similar to that of protons. However, it soon became apparent that μ mesons are produced by weak interactions, not by strong interactions. Protons are discovered later, and protons decay into μ mesons.
In the 1930s, people realized that things were getting chaotic and there was an apparent need to classify and reconcile particles. In 1936, people began to see more particles, and Heisenberg realized that neutrons and protons were very similar. Therefore, they argue that just as an electron has two spins, there should be another internal symmetry that links neutrons and protons.
There was no progress in the early 1940s. However, things picked up in 1947. It was a wonderful year in physics. People began to rethink the natural world and discovered the Lamb shift. The Lamb shift was originally a strange difference in the energy levels of hydrogen 2S and 2P, which physicists thought should be the same. However, it has been observed that there are slight differences in energy, which cannot be explained by the quantum mechanics of Dirac's equations. Lamb first measured the Lamb displacement in the Lamb-Rithamford experiment in 1947.
The Lamb shift has sparked a new wave of research on quantum electrodynamics. It turns out that the way to explain this difference is quantum fluctuations in a vacuum that were not previously included in quantum field theory due to divergences that have arisen in mathematics. However, Schwenger, Feynman, Dyson, and Asahina used re-normalization techniques to overcome this and successfully explained the phenomenon. The alignment of theory with the experiment is a huge victory for quantum field theory. Most importantly, at this time, Yukawa's π meson was also discovered.
This is the first part of a series of Standard Model histories, so stay tuned for the next one.